How Do Schwann Cells Repair Damaged Nerves
Abstract
Since Schwann cells (SCs) support axonal growth at development as well as afterwards peripheral nervus injury (PNI), developing SCs might be able to promote axon regeneration after PNI. The purpose of the current study was to elucidate the adequacy of developing SCs to induce axon regeneration subsequently PNI. SC precursors (SCPs), immature SCs (ISCs), repair SCs (RSCs) from injured nerves, and non-RSCs from intact nerves were tested by grafting into acellular region of rat sciatic nerve with beat injury. Both of developing SCs completely failed to support axon regeneration, whereas both of mature SCs, especially RSCs, induced axon regeneration. Further, RSCs only not SCPs promoted neurite outgrowth of adult dorsal root ganglion neurons. Transcriptome assay revealed that the gene expression profiles were distinctly unlike betwixt RSCs and SCPs. These findings indicate that developing SCs are markedly dissimilar from mature SCs in terms of functional and molecular aspects and that RSC is a feasible candidate for regenerative prison cell therapy for PNI.
Introduction
In contrast to the poor regenerative chapters of the central nervous organization (CNS), the peripheral nervous system can regenerate after injury. Nevertheless, the clinical event is non e'er satisfactory especially in instance of proximal injury or large defect1,two. In addition, autologous nerve graft (ANG) has been a aureate standard since the early 20th century for the reconstruction of nerve injury with big defects,i,3,iv, still accompanying several issues such equally donor site morbidity, express supply, and motor-sensory and size mismatchfour,five. Due to the recent advancement of biomaterials, new treatment options such equally a constructed scaffold or decellularized allograft became clinically available to overcome the issues derived from ANGhalf dozen,vii. Withal, their axon-promoting furnishings are withal non comparable to ANG. Accordingly, a therapy superior to ANG has been desired for decades.
Recently, cell therapy has been attracting much attention every bit a potent therapy in multiple organs8,9,10,eleven,12,13. Considering a jail cell type for the graft for peripheral nerve injury (PNI), peripheral glia, Schwann cell (SC), is a potent candidate because the graft of SCs enhanced axon regeneration after PNI14,15,16,17,xviii,xix,20 and regenerating axons always accompany SCs21. Further, with the rapid growth of stalk jail cell research, novel SC-like cells have been developed as a new graft material. They have differentiated from various kinds of stem cells22,23,24,25,26 or fibroblasts with direct reprogramming technique27.
Interestingly, the SCs used for the study of axon regeneration after PNI has been always mature cells, and developing SCs were rarely tested, although they involve axonal evolution and finally differentiate into mature SCs28. This is a sharp contrast to the written report of CNS injury where developing merely not mature neural cells are the main jail cell types used for therapeutic purposes including axon regeneration10,29,30. Accordingly, it is reasonable to assume that the SCs at developmental stages can also promote axon regeneration or might exist superior to mature SCs as a graft prison cell source for PNI.
Importantly, it was previously idea that SCs dedifferentiated into the phenotype of developing SCs after injury to support the reparative procedure, based on the ascertainment that so many gene expressions of denervated fretfulness were shared with developing nerves, including loss of myelin and upregulation of developing SC markers31,32,33. Recently, accumulated evidence replace this idea with a new i that injury response reprograms mature SCs and converts them to repair SCs (RSCs) specializing in the repair procedure and that they are substantially different from developing SCs28,31. Withal, this recent evidence does not necessarily deny the reparative potential of developing SCs. Farther, information technology remains unclear well-nigh the actual departure of the molecular profiles between RSCs and developing SCs.
Therefore, the purpose of the current report is to elucidate the therapeutic potential of developing SCs by investigating axon-promoting effects after PNI and by clarifying their molecular profiles, compared to RSCs. The current written report demonstrates that, unlike CNS, developing SCs accept no capacity to support the regeneration of adult axons after PNI, that developing SCs are distinctly unlike from RSCs in molecular and functional aspects, and that RSC is a stiff candidate every bit a graft prison cell type for axon regeneration therapy later on PNI.
Results
Mature SCs but not developing SCs supported axon regeneration
SCs develop from neural crest cells, differentiate to SC precursors (SCPs) around E14 (Fig. 1a) and and then to young SCs (ISCs) effectually E18 (Fig. 1b)34,35. Accordingly, nosotros tested the axon-promoting effect of SCPs and ISCs by comparing two types of mature SCs, RSCs, and nonRSCs prepared from injured and intact fretfulness, respectively (Fig. 1c). Characterization of prepared SCs demonstrated that SC marker, Sox10, was expressed in all types of SCs but another SC marker, S100β, is not expressed in SCPs (Fig. 1d)36,37,38. Based on Sox10 immunolabeling, the purity of prepared SCs were 87%, 95%, 95%, and 92% for SCPs, ISCs, nonRSCs, and RSCs, respectively (Fig. 1d, due east). When examining immature markers, not but developing but also mature SCs limited nestin and Sox2 (Fig. 1d, e, Supplementary Fig. one), suggesting that dissociated SCs beginning expressing these markers39,xl,41.
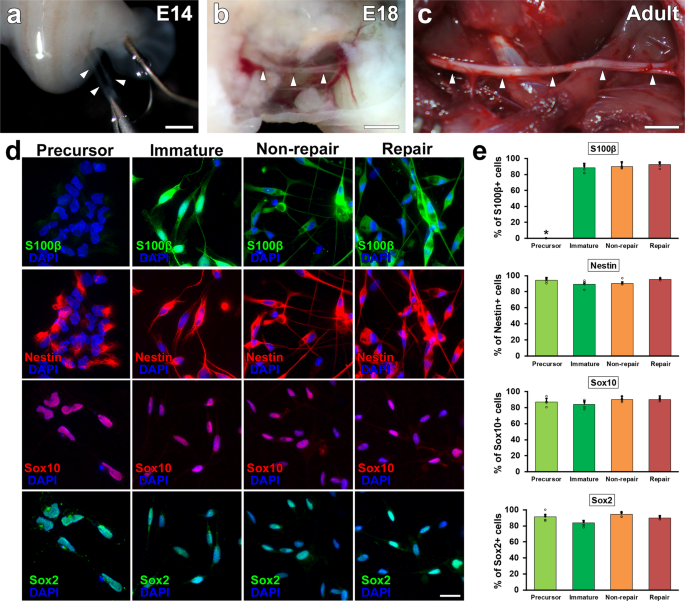
a–c Outlook of sciatic nerves at different developmental stages. SCPs, ISCs, and not-RSCs were harvested from intact sciatic fretfulness at E14 (a), E18 (b), and postnatal x–12 weeks (c). Arrowheads betoken sciatic nerves. Scale confined: 1 mm (a, b), 5 mm (c). d Immunolabeling of cultured SCs against S100β, Nestin, Sox10, and Sox2. Regardless of SC types, most cells express immature marker, Nestin and Sox2, and SC marker, Sox10. In contrast, some other SC mark, S100β is absent-minded only in SCPs. Scale bars: xx µm. eastward Quantifications of % of immunolabeled SCs confronting S100β, Nestin, Sox10, and Sox2. Five samples per grouping. At least 50 cells were counted in each sample. *P < 0.05 vs. SCP, ane-fashion ANOVA with the Tukey–Kramer test. Mistake bars represent the SEM.
Two million of each blazon of cells in x µl phosphate-buffered saline (PBS) were grafted into 25 mm-long acellular regions with beat out injuriesxx. Equally a negative command, 10 µl of PBS lone was injected. Ii weeks after cell grafts and injuries, rats were perfused, followed by an immune-histological cess. Grafted cells survived well and filled up acellular regions in all groups (Fig. 2a, b). Quantification of RFP expressing grafted cells showed that the SCPs and the ISCs groups had greater survival than the RSCs and the nonRSCs groups in proximal regions (Fig. 2c). The grafted ISCs had significantly more Ki-67 positive cells than the RSCs and the nonRSCs in all quantified areas (Fig. 2d, east), whereas the grafted SCPs showed more Ki-67 positive cells than the RSCs and the nonRSCs only in proximal regions. This reduced proliferation of SCPs in the distal regions could be attributed to the fact that the proliferation of SCPs depends on neuregulin released from growing axons42. Regarding axon regeneration, the RSCs induced the greatest regeneration among all tested cells at every quantified point (Fig. 3a, b). Next was the nonRSC, which induced significantly more than axon regeneration than the other 3 groups at seven.5 mm indicate from the proximal injury site (Fig. 3b). In contrast, developing SCs did not demonstrate any axon-promoting upshot at all, when compared to no prison cell graft. Further analysis about a regeneration unit, which is a complex of regenerating axon and an SC providing a substrate for growth43,44, revealed that a significantly higher % of the RSCs formed regeneration units than other cells (Fig. 4a, b), suggesting the directly effect of the RSCs on axon regeneration. These results indicate that mature SCs but not developing SCs tin promote axon regeneration, and the RSCs possess the superior property to directly support axon regeneration by making regeneration units among tested four types of SCs.
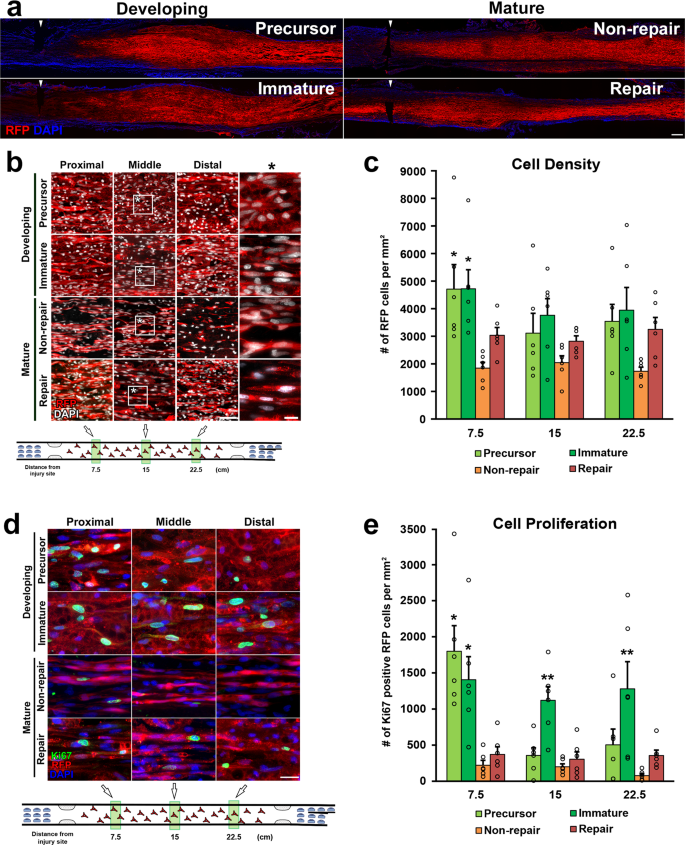
a Low magnification images of longitudinal sections effectually the proximal injury sites 2 weeks subsequently SCs grafts. RFP expressing SCs of all types fulfill injured nerves. Arrowheads indicate the proximal injury sites marked by small cuts. Left is proximal. Scale bar, 500 µm. b RFP expressing grafted SCs at proximal, centre, and distal ane-tertiary of graft areas. Scale bar, 100 µm. *: High magnification paradigm of the boxed surface area. Scale bar, x µm. c Quantification of the density of grafted cells at seven.five, xv, and 22.five mm points from the proximal injury site. At the vii.5 mm point, SCPs and ISCs graft subjects demonstrate statistically college densities of RFP expressing cells than RSCs and non-RSCs subjects. Half-dozen rats per grouping. *P < 0.05 vs. northward RSC and non-RSC, One-way ANOVA with the Tukey–Kramer test. Error bars represent the SEM. d High magnification images of Ki67 immunolabeling at the proximal, middle, and distal one-third of graft areas. Scale bar, 20 µm. e Quantification of the density of Ki67 immunolabeled RFP expressing graft cells at seven.5, 15, and 22.5 mm points from the proximal injury site. Developing SCs take more proliferating than mature SCs afterwards grafting. *P < 0.05 vs. non-RSC and RSC, **P < 0.05 vs. other three groups. One-mode ANOVA with the Tukey–Kramer test. Fault bars represent the SEM.
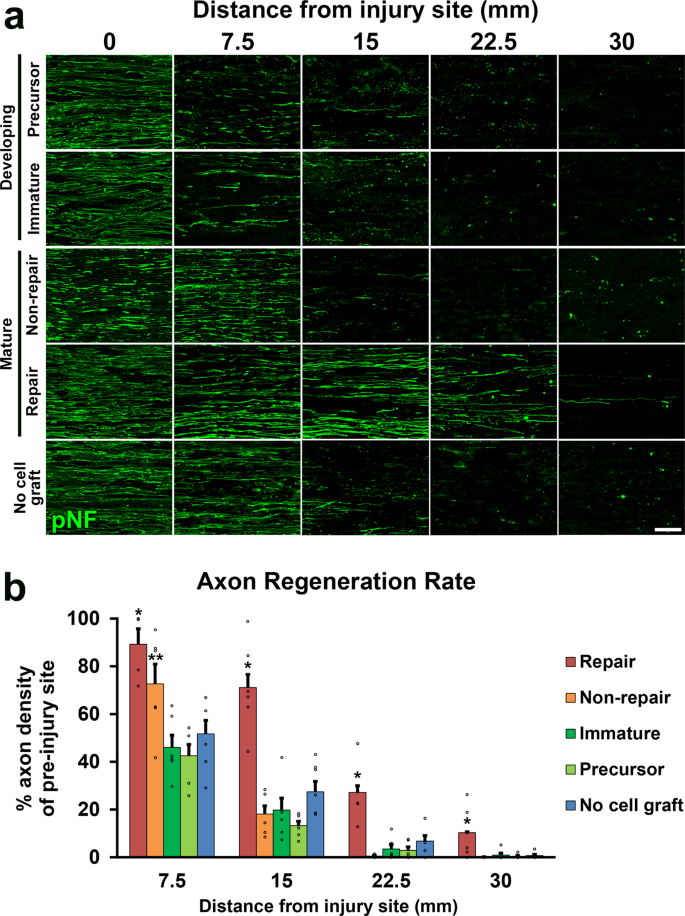
a Representative images of regenerating axons labeled by pNF in 5 groups, which are graft of SCPs, ISCs, non-RSCs, RSCs, and no cell graft. RSCs grafts induced substantial axon regeneration. Left is proximal. Scale bar, l μm. b Quantification of regenerating axons. RSCs grafts demonstrated the greatest axon-promoting effect amongst all groups. Non-RSCs grafts revealed statistically more axons than SCPs graft, ISPs graft, and no cell graft. 6 rats per group. *P < 0.05 vs. others, **P < 0.05 vs. ISCs, SCPs, and no cell graft, one-way ANOVA with the Tukey–Kramer test. Error bars represent the SEM.
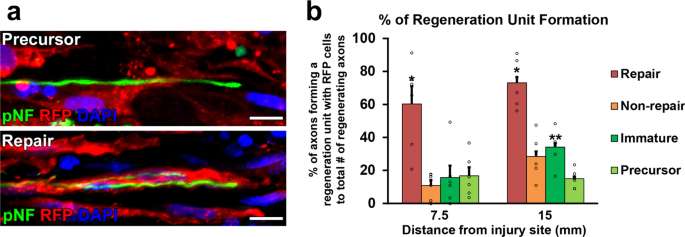
a High magnification images of regenerating axons (pNF, green) and grafted cells (RFP, red). Arrowheads point regenerating axons, which are tortuous and have growth cone-like morphology. The upper image is a representative instance of the lack of a regeneration unit formation by grafted RFP expressing SCPs. RFP expressing SCPs have no association with a regenerating axon. In contrast, the lower image shows that RFP expressing RSCs form a regeneration unit with regenerating axons. Scale bars, 10 μm. Left is proximal. b Quantification of regeneration unit of measurement germination. Percentages of axons forming a regeneration unit with RFP+ cells to total # of regenerating axons were quantified. Grafted RSCs have a great capacity to grade regeneration units. Half dozen rats per group. *P < 0.05 vs. others. **P < 0.05 vs. SCPs. One-fashion ANOVA with the Tukey-Kramer test. Mistake bars stand for the SEM.
RSCs tin can promote neurite outgrowth of adult DRG neurons more effectively than SCPs
To confirm the finding that only mature simply non developing SCs can promote the growth of injured adult axons, we further analyzed the event of SCs on neurite outgrowth of co-cultured DRG neurons. Nosotros compared SCPs with RSCs, because SCPs were expected to promote axon regeneration more than ISCs due to their immaturity28 and association with growing axons45. Ii kinds of DRG neurons were prepared from E14 and adult fourth dimension points, and they were cocultured with SCPs or RSCs (Fig. 5a). Later on 24 h of co-civilization, embryonic DRG neurons elongate their neurite well regardless of presence or type of SCs (Fig. 5b, c–due east), indicating that the intrinsic growth holding of embryonic DRG neurons is robust and that their neurite outgrowth is independent of extrinsic factors such molecules provided by cocultured SCs. In contrast, adult DRG neurons rarely elongate their neurites without cocultured SCs (Fig. 5b, f–h), and the cocultured SCs enhanced neurite outgrowth significantly. The effect of RSCs was the most, which was nigh 97% greater than that of SCPs in the longest neurite length (Fig. 5b, f–h). This result indicates that the growth holding of adult DRG neurons is poor but mature SCs tin can support their neurite outgrowth effectively more developing SCs.
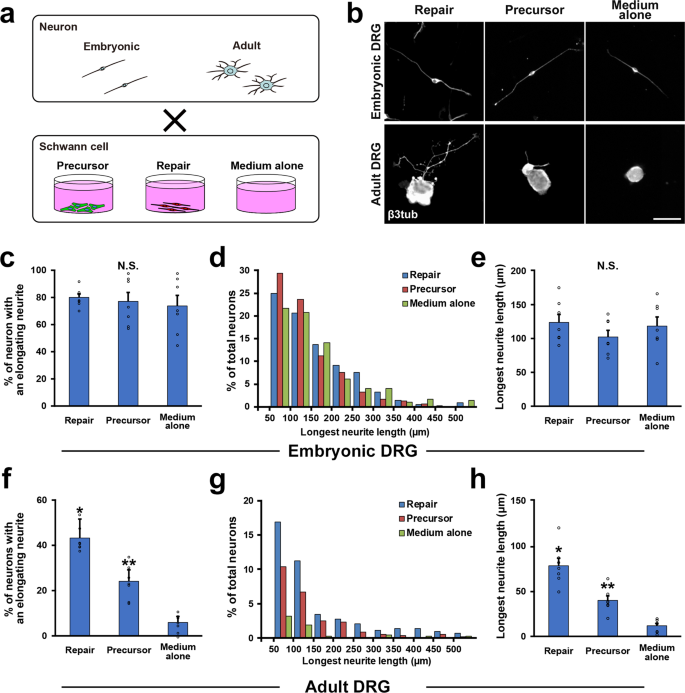
a Schematic diagram of co-culture of DRG neurons and SCs. b Representative images of neurite outgrowth of DRG neurons in each condition. Immunolabeling with β3 tubulin depicted neurite elongation. Upper images are embryonic DRG neurons and neurite outgrowth of all iii groups is comparable in length. Lower images are adult DRG neurons, showing a neuron co-cultured with RSCs elongated its neurite longer compared to neurons in other conditions. Scale bar, 50 μm. c Quantification of % of elongating embryonic DRG neurons. No statistical difference was detected among groups. Vii samples per grouping. At least 50 neurons were calculated in each sample. d Percentage of embryonic DRG neurons with the longest elongating neurite in the categories shown. At that place is no credible distribution design among groups. eastward Quantification of the longest neurite of embryonic DRG neurons. There is no statistical difference amid groups, demonstrating that the presence of co-cultured SCs does not affect neurite outgrowth of embryonic DRG neurons. f Quantification of % of elongating adult DRG neurons. RSCs stimulated neurite outgrowth of adult DRG neurons significantly more than SCPs, and the next is SCPs compared to the medium alone. 7 samples per grouping. At least 50 neurons were calculated in each sample. g Percentage of developed DRG neurons with the longest elongating neurite in the categories shown. h Quantification of the longest neurite of developed DRG neurons. RSCs promoted the length of neurite outgrowth of adult DRG neurons most among groups and the adjacent is SCPs compared to medium alone. *, **P < 0.05 vs. others. I-way ANOVA with the Tukey–Kramer test. Error bars represent the SEM.
Gene expression profile was distinctly unlike betwixt RSCs and SCPs
To explore the molecular mechanisms underlying the marked difference of axon-promoting effects betwixt the RSCs and the SCPs, nosotros performed their transcriptome analysis by RNA-seq. Transcript expression levels were significantly different in 6804 genes (p < 0.01), and 1933 genes were more than four-folds changed (p < 0.01, log2FC > 2), clearly indicating that gene expression profiles of the RSCs and the SCPs are distinctly unlike (Fig. 6a, b). Additional analysis by gene ontology (Get) showed that several biological features were as well significantly unlike. The meridian vii genes enriched in the SCPs were development and morphogenesis-related ones but not repair and inflammation-related ones, whereas these in the RSCs were inflammatory response, cytokine production, and regulation of immune response (Fig. 6c), that are known to involve in repair processes afterwards PNI44,46,47,48. Kyoto Encyclopedia of Genes and Genomes (KEGG) pathway analysis as well identified axon guidance equally to the most upregulated pathway in the SCPs (Fig. 6d), equally expected in glial cells at the developing stage. Other well-known pathways in developing SCs, Hippo, and Wnt signaling pathways were also upregulated28,49,50. In contrast, in the RSCs, upregulated pathways were osteoclast differentiation, TNF signaling, and Toll-like receptor signaling pathways (Fig. 6d), which are related to the AP-i(Jun/Fos) transcription cistron that is critical for the repair office of the RSCs51,52. Furthermore, a considerable number of genes related to basement membrane components such as collagens, laminins, and fibronectins53 are differently expressed (Supplementary Fig. ii). These findings indicate that there are rarely shared molecular features between the SCPs and the RSCs and that the molecular profile of the SCPs is exclusively related to development but not the repair procedure to which the molecular profile of the RSCs is mainly related. Lastly, nosotros quantified the secretion of two axon-promoting neurotrophic factors, nerve growth factor (NGF) and brain-derived neurotrophic factor (BDNF)54 in the SCPs and the RSCs. Notably, the RSCs produced significantly more NGF and BDNF than the SCPs (Fig. 7), supporting the finding of the marked difference of axon-promoting effect of these SCs at the protein level.
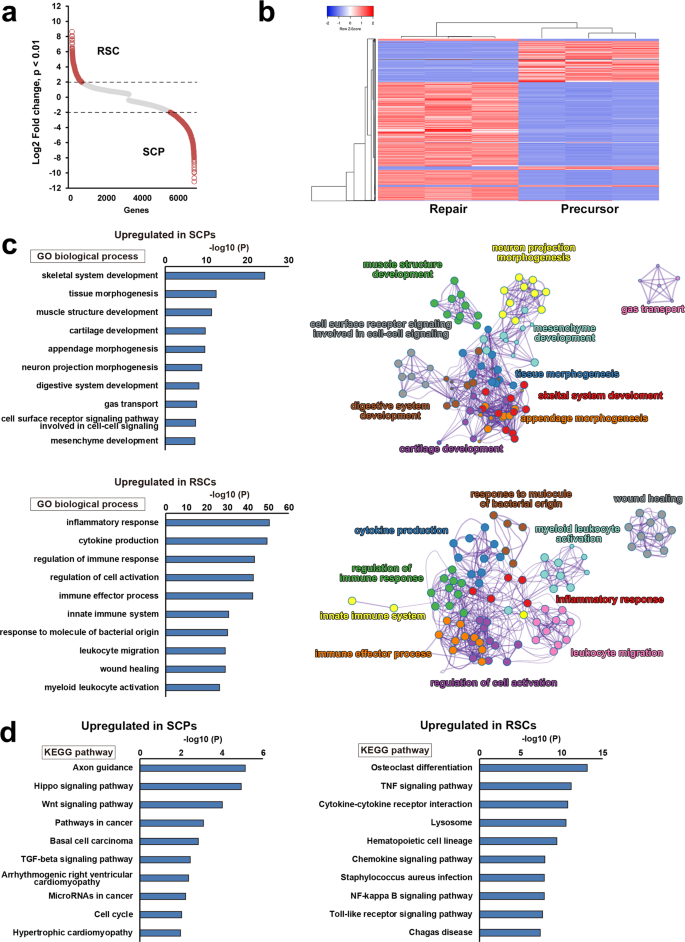
a Scatter plot analysis for transcript expression levels of significantly upregulated or downregulated genes in RSCs and SCPs. Red circles indicate genes with Log2 fold change over ii or nether −2. Transcript expression levels were significantly different in 6804 genes (p < 0.01), and 1933 genes were more than than 4-folds changed (p < 0.01, log2FC > 2). b Heatmap of gene expressions in RSCs and SCPS. They revealed a high degree of similarity between the samples in each grouping, and gene expression profiles are distinctly different betwixt RSC and SCPs. Ruby-red and blue betoken the highest and lowest relative levels of gene expression. c Pinnacle x enriched Go terms of biological procedure and their cluster visualization in SCPs (upper row) and RSCs (lower row). Development-related terms are upregulated in SCPs, whereas inflammation-related terms are upregulated in RSCs. Cluster annotations are shown in each clusters' color. d KEGG pathway analysis in SCPs (left) and RSCs (right). Biological terms are distinctly different between these two cells.
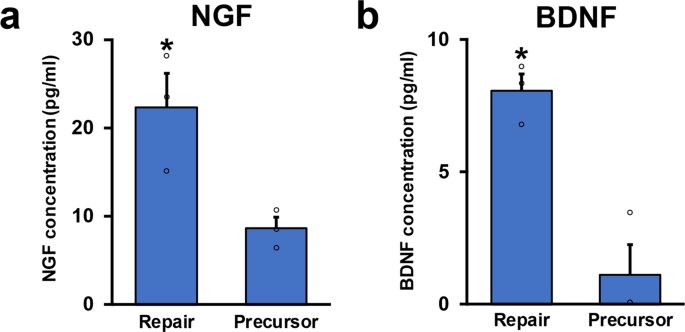
a NGF concentrations of conditioned medium of RSCs and SCPs. RSCs secrete more than twofold of NGF than SCPs exercise. Iii samples per group. *P < 0.05; Student's t-examination. Mistake bars correspond the SEM. b BDNF concentrations of conditioned medium of RSCs and SCPs. RSCs secrete more than fivefold of BDNF than SCPs practise. Three samples per group. *P < 0.05; Pupil'southward t-test. Mistake bars represent the SEM.
Word
The electric current written report demonstrated that the capacity of SCs to promote axon regeneration after PNI totally depended on their developmental stage. Developing SCs such as SCPs and ISCs failed to promote axon regeneration, whereas mature SCs could induce axon regeneration. This is quite notable because in that location are several reasons to expect developing SCs to promote axon regeneration after PNI. Commencement, many literatures report that developing cells have a therapeutic effect on tissue repair or regenerationeight,nine,12,55,56,57. 2nd, in CNS injury models, grafts of developing cells induced axon regenerationx,30,58. 3rd, when developing neurons extend their axons, neighboring immature glial cells support them38,45,59,60. Fourth, because developing cells are more often than not more proliferative and resilient than mature cells, they could survive better when grafted. Lastly, developing cells differentiate in the graft environs. Since they are plastic, they might prefer the injured environment and differentiate into more reparative phenotype34,61,62,63,64,65. Regardless of these reasonable facts, developing SCs has no axon promoting effect at all subsequently PNI, clearly indicating that developing SCs are specialized in supporting developing axons but non injured adult axons and that the extrinsic mechanism of regenerating axons differ from that of developing axons.
Developing SCs showed interesting behavior subsequently grafting in the current study. The number of survived SCPs was smaller in more distal areas than the proximal area (Fig. 2c). In development, the survival of SCPs but not ISCs depends on secreted molecules such as neuregulin66 from neighboring growing axons42,67. Appropriately, this interesting behavior is causeless to be the result that regenerating axons secrete soluble molecules stimulating SCPs like developing axons68,69 and that merely SCPs close to regenerating axons can survive after grafting by receiving stimulation of secreting molecules from axons. This finding suggests that prepared SCPs nonetheless retain the initial phenotype even after grafting into the injured environs.
Accumulated evidence shows that axon regeneration requires both intrinsic and extrinsic mechanismslxx,71,72,73,74. Based on the consequence of the co-culture experiment (Fig. 5) and others43, developed DRG neurons need extrinsic support from SCs for neurite outgrowth. In contrast, developing DRG neurons demonstrated substantial neurite outgrowth independently from co-cultured SCs (Fig. 5c, east), suggesting that developing DRG neurons have a bully intrinsic growth capacity and require no extrinsic support from SCs. Indeed, developing SCs contribute to axonal sorting and maintenance but non to axonal extensionsixty,75, supporting this observation. The extrinsic mechanism of axon regeneration consists of secreted factors as well as contact-mediated factors43,76. Regarding the former factors, SCPs do not secrete a comparable amount of neurotrophic factors to RSCs (Fig. 7). Most the latter factors, their expression contour of collagens, laminins, and fibronectins, which are major extracellular matrix (ECM) molecules associated with basement membrane53,77 was quite different from that of RSCs (Supplementary Fig. two), and developing SCs couldn't form many regeneration units (Fig. 4b)78. Although the exact office of ECM molecules on axon regeneration is non fully understood, some type of collagen and laminin is reported to have critical roles in axon regeneration79,fourscore. The obtained results propose that developing SCs generate less ECM necessary for axon regeneration than mature SCs, and these facts explain at least partially the reason why developing SCs failed to promote axon regeneration.
In the electric current written report, nosotros employed a 2-week time point to assess the axon promoting effects of grafted cells, because this time point was originally defined as optimal to compare the axon promoting effects in this experimental model20. At later fourth dimension points, this model has less sensitivity to find the difference of axon promoting furnishings, because more axons regenerate and more than host SCs migrate into acellular region. Regarding the therapeutic effects of each SCs, we did not perform a functional assessment, because information technology does non necessarily reflect the ability to promote axon regeneration of grafted SCs. Functional recovery later PNI requires axon regeneration and then remyelination. However, the chapters of each SCs to promote remyelination remains unclear. Myelination-related genes are differentially expressed in each SCs81,82, indicating that their remyelinating effects after PNI would vary. In addition, the extent of proliferation is different among each SCs (Fig. 2e). Although the ability of grafted SCs to promote axon regeneration is critical, multiple aspects of SCs will bear upon therapeutic effects induced by the graft of SCs.
The current written report revealed that molecular profiles of SCPs and RSCs were distinctively different (Fig. 6), supporting the recent idea that RSCs are non dedifferentiated SCs like developing SCs35,44. In item, SCPs upregulate guidance molecules and pathways related to development, whereas RSCs upregulate cytokine product, inflammation-related molecules, and pathways, exactly reflecting the environs where SCs located (Fig. 6). SCPs are surrounded by immature cells and lilliputian inflammation, whereas RSCs are in the center of inflammation consisting of debris and immune cells47,83,84,85,86. This molecular comparison data provides the footing for analyzing the characteristics of RSCs in addition to the previous analysis48.
Based on the current findings, RSC is a potent candidate as a graft textile for cell therapy of PNI, especially compared to nonRSCs. However, previous attempts of SC grafts for PNI used nonRSCs and there was piffling information on their molecular and cellular profilesxiv,16,17,18. SCs were prepared from the patient'south intact sural nerve or intact rat sciatic nerve and expanded several times. In those studies, if RSCs were used instead of nonRSCs, more regeneration could take been induced. For clinical translation, it is necessary to establish the protocol to generate RSCs from nonRSCs and maintain their therapeutic effects. Ideally, the generation of RSCs from pluripotent stem cells or allogenic cells is suitable for a applied clinical application, since no sacrifice of the nerve is required.
In rodents, SCs start to modify their transcriptional design into repair phenotype in several hours afterwards PNI48, and by 1 to four weeks after PNI, they dramatically change its morphology through demyelination, elongation, and branching87. These steps undergo smoothly in young mice, withal, in aged mice, phenotypic change of SCs is impaired, resulting in poor axon regeneration72. Based on these findings with the current findings, dispatch of phenotypic change from nonRSCs into RSCs is a therapeutic target for PNI.
In conclusion, RSCs demonstrated the greatest axon-promoting effects among 4 types of SCs in different developmental stages. Unlike CNS, developing glia had no significant effects on axon regeneration. Time to come therapeutic strategies include implantation of RSCs with an establishment of conversion method from nonRSC to RSC and an identification of fundamental molecules expressed by RSCs for axon regeneration.
Methods
Animals
Adult LEWIS rats (Wild-type, Charles River Laboratories Nihon, Inc.) were used in all experiments. Their body weight ranged from 155 to 211 g with an average of 185 thou. Graft cells were prepared from syngeneic adult LEW-Tg (Gt(ROSA)26Sor-DsRed*)7Jmck rats that ubiquitously express the DsRed monomer driven by the gene trap ROSA 26 promoter, supplied past the National BioResource Project (Kyoto University, Kyoto, Japan). The study protocol was approved by the local ethical commission of Hokkaido Academy. Animals had complimentary admission to food and h2o throughout the written report. For animal anesthesia, a mixture of ketamine (75–100 mg/kg, KETALAR®, Daiichi Sankyo Propharma Corporation, Tokyo, Japan) and medetomidine (0.v mg/kg, DOMITOR®, Orion Corporation, Espoo, Finland) was administered by intraperitoneal injection.
SC preparation
All grafted cells used in this report were prepared from transgenic LEWIS rats that ubiquitously expressed RFP and that were syngeneic to wild-type LEWIS rats. SCPs and ISCs were harvested using a modified protocol described previously38,88. In cursory, E14 and E18 Embryos were harvested from fourth dimension-mated pregnant females, and bilateral sciatic fretfulness and brachial plexus were dissected using fine forceps (Fine Science Tools, No.11252-202). Then, dissected nerves were transferred to an enzymatic digestion medium containing 0.1% collagenase I (Sigma-Aldrich) and 0.125% trypsin in Dulbecco'due south modified Eagle medium (DMEM)/Ham's F-12 (DMEM/F12, Wako, Osaka, Japan). Afterward incubation for thirty min at 37 °C, nerves were mechanically dissociated by pipetting 30 times in a ane ml SC civilisation medium, which consists of DMEM/Ham'due south F-12 supplemented with x% fetal bovine serum (FBS), one% GlutaMAX (Thermo Fisher Scientific, Waltham, MA), and ane% penicillin-streptomycin (PS, Thermo Fisher Scientific). RSCs and non RSCs were prepared from intact sciatic fretfulness and distal segments of transected sciatic fretfulness of 8–x week one-time rats according to a modified protocol89. Briefly, sciatic nerves running from the sciatic notch or the transection site to the end of the femur were dissected, cut into ane- to ii-mm pieces using micro-scissors later on removal of the epineurium, and transferred to enzymatic digestion medium containing 1% collagenase I and 0.125% trypsin in DMEM/F12. Later incubation for one h at 37 °C, tissues were mechanically dissociated by pipetting 30 times in a 1 ml SC culture medium. To remove myelin debris, prison cell intermission of non RSCs were resuspended in 10 ml SC culture medium, and mixed with 8 ml Percoll plus (GE Healthcare, Chicago, IL), 2 ml 10× HBSS (Wako, Osaka, Japan), 10 ml 1× HBSS, and centrifuged thirty min in 4 °C using fixed bending centrifuge rotors. Ten ml solution at the bottom was gently taken and done with 1× HBSS. Lastly, to remove adhesive cells such as fibroblasts and macrophages, cells were seeded in 75 cm2 non-coated flasks for 30 min, and cells floating in culture medium were used for experiments. Cell viability, which was assessed with trypan blueish (Life Technologies, Chiliad Island, NY), was inside the range of 92–99% for all jail cell preparations. For characterization, cells were cultured on poly-l-lysine (PLL, Sigma-Aldrich) and laminin (Sigma-Aldrich) coated 24-well plates at a density of 1.0 × tenv cells/cm2 with the SC civilisation medium. For grafting and RNA extraction, prepared cells were resuspended in PBS (Thermo Fisher Scientific). For coculture experiment or ELISA analysis, cells were resuspended DMEM/F12 supplemented with two% B27 (Thermo Fisher Scientific), Heregulin (20 ng/ml, Peprotech), ane% GlutaMAX, and i% PS.
Surgical procedures
A recently adult experimental model defended to assessing the efficacy of grafted cells on axon regeneration was used20. Briefly, the sciatic nervus was exposed and received two crush injuries made past micro-musquito forceps. The proximal injury was just distal to the sciatic notch, and the distal injury was 25 mm distal to it. For making decellularization area, this 25-mm long area between two injury sites was subject area to repeated frozen by liquid nitrogen and spontaneous thaw at room temperature v times. To mark the injury site, a stay suture was placed at the epineurium merely next to the injury site. A total of 30 rat sciatic fretfulness were divided into the post-obit five groups, (1) SCP grafts, (2) ISC grafts, (three) nonRSC grafts, (4) RSC grafts, and (5) no prison cell graft. One million cells in x µl PBS were grafted into the decellularized area by four injections through a 34 gauge needle of NanoFil syringe (Globe Precision Instruments, Sarasota, FL) in jail cell grafts groups, and ten µl PBS alone were injected using the aforementioned method in the no cell graft group.
Coculture of DRG neurons and SCs
Embryonic DRGs and adult lumber DRGs were dissected from E14 and ten–12 weeks onetime wild-blazon LEWIS rats. Embryonic DRGs were incubated in 0.1% collagenase type XI(Sigma-Aldrich) for 1 h at 37 °C, and adult DRGs were incubated in 0.5% collagenase type Xi solution for i h at 37 °C. After enzymatic digestion, they were mechanically dissociated past pipetting thirty times in a 1 ml medium, consisting of DMEM/Ham's F-12 supplemented with 2% B27, 1% GlutaMAX, and ane% PS.8,9,ninety Embryonic and adult DRG neurons were cocultured with SCPs or RSCs. SCs were seeded at a density of 5.0 × x4 cells/cm2 on the 48-well plate coated with PLL and laminin. 3 hours later, 5.0 × 10three DRG neurons/cm2 were placed in the wells with the neuron culture medium. 20-4 hours after, cells were stock-still with 4% paraformaldehyde (PFA, Nacalai Tesque Inc., Kyoto, Japan) in 0.ane% phosphate buffer (PB). Neurite outgrowth was quantified as described previously43. Images were taken by an all-in-one fluorescent microscope (BZ-X710, Keyence, Osaka, Nihon) using a 20× objective lens. Neurites were traced and measured past ImageJ91 with plugin software, NeuronJ, as described previously71,92. To avoid the effect of neuron-neuron interaction, if their neurites touched neurites of other neurons, these neurons were excluded from the analysis. L micrometer or longer neurite was defined equally an elongating neurite93. At least 50 neurons were randomly selected per well, and the % of neurons with elongating neurites and the averages of longest neurites were calculated. Experiments were repeated three times and a total of six wells per condition were obtained.
Immunohistochemistry and immunocytochemistry
For immunohistochemistry, sciatic nerves were dissected later on perfusion with 4% PFA in 0.1% PB, followed by overnight fixation with four% PFA at 4 °C. On the following mean solar day, nerves were transferred into 30% sucrose in 0.1% Lead and stored until sectioning. When sectioning a nervus, a pocket-sized cutting was made at the injury site to marking the injury site. Nerves were sagittally sectioned using cryostat at 10-μm intervals and directly mounted on x slides in club. For immunocytochemistry, cultured cells were stock-still with four% PFA in 0.1% Pb for 15 min. Fixed cells or sections were incubated overnight with master antibodies confronting RFP (1:200, goat from Sicgen, Portugal), pan neurofilament (pNF, 1:1000, mouse from Biolegend, San Diego, CA), S100β (1:200, rabbit from Abcam, Cambridge, UK), Ki67 (1:500, rabbit from GeneTex, Irvine, CA), β3 tubulin (1:1000, rabbit and mouse from Biolegend), Nestin (1:500, mouse from BD Bioscience, Franklin Lakes, NJ), Sox2 (one:500, rabbit from Merck Millipore, Burlington, MA), and Sox10 (1:100, caprine animal from R&D systems, Minneapolis, MN) at iv °C. Then, after washing with Tris-buffered saline, sections were incubated in Alexa 488, 594, or 647 conjugated to ass secondary antibodies (i:yard, Jackson Immunoresearch, West Grove, PA) and DAPI for 1 h at room temperature.
Quantification
3 consecutive sections from the middle part of the nerve were used for quantification of in vivo studies. The total number of RFP expressing cells were identified by the presence of DAPI surrounded by RFP immunoreactivity at high magnification image, quantified in a 100-µm wide region at three points, which were 7.5, 15, 22.v mm distal to the proximal injury site, and divided past the full quantified surface area as the density of grafted cells. For quantifying the proliferating grafted cells, the RFP and Ki67 double-immunoreactive cells were counted in the aforementioned manner. Axon regeneration rate was quantified as described previouslyxx. Briefly, lines perpendicular to sections were set at points 7.five, 15, 22.5, and 30 mm distal and merely proximal to the injury site. The numbers of pNF-labeled axons crossing each line were quantified. For normalization, the sum of the axon numbers of three sections was divided past the sum of the length of each line equally an axon density. To calculate the per centum of axon regeneration, the axon density of each betoken was divided by the density at the uninjured site, which was 1.5 mm proximal to the injury site. To evaluate the iii-dimensional relationship between axons and grafted cells, triple stained sections for pNF, RFP, and DAPI were imaged at points 7.5 and xv mm distal to the injury site in which regenerating axons are observed in all samples with a confocal laser microscope (FV-thou, Olympus, Tokyo, Japan) at the 1000× magnification. The number of axons that have a close association with graft cells was divided past the total number of axons as the % of regeneration unit formation.
RNA-seq
RNA was extracted from SCPs and RSCs using TRIzol (Thermo Fisher Scientific, Waltham, MA) and RNeasy plus mini kit (Qiagen, Netherland) using the manufacturer's instructions. Full RNA integrity and quality were assessed with Aglient 2100 bioanalyzer (Aglient Technologies, Santa Clala, CA, The states), and samples over 1 μg which had an RNA integrity number greater than 7 were used for RNA-seq. Each library was generated using the TruSeq Strandard mRNA Sample Preparation Kit (Illumina, Inc., San Diego, CA, The states), and paired-stop reads (100 bp) were obtained past Illumina NovaSeq 6000 (Illumina, Inc). Reads were mapped by alignment to rattus norvegicus genome rn6 and analyzed by TopHat, Gage links, and Cuffdiff94 Genes with p value < 0.01 and log2FC > 2 were divers as differentially expressed genes (DE genes). GO and KEGG (Kyoto Encyclopedia of Genes and Genome) pathway analyses were performed using DAVID (https://david.ncifcrf.gov/) databases and metascape (https://metascape.org/gp/index.html#/main/step1). Heatmap was fabricated using Heatmapper (http://www.heatmapper.ca/expression/). Clusters of biological processes were visualized past prefuse force-directed layout using Cytoscape (https://cytoscape.org).
Enzyme-linked immunosorbent assay (ELISA)
SCPs and RSCs were seeded at a density of ii.0 × x5/well on the 24-well plate coated with PLL and laminin and cultured in 1.5 ml of culture media mentioned above. Concentrations of NGF and BDNF were measured using Rat NGF/BDNF ELISA kit (Abcam, Cambridge, Great britain) post-obit the manufacturer's instruction (N = 3/group). The absorbance was read at 450 nm using Benchmark-Plus Reader (BIO-RAD, CA).
Statistical assay
The normality of the information distribution was assessed with the Shapiro–Wilk exam. Multiple-group comparisons were made with Kruskal–Wallis analysis of variance and the Tukey–Kramer test, and two-group comparisons were made with the unpaired 2-tailed Pupil's t-test. All analyses were performed with JMP Pro 14.0 software (SAS Institute, Cary, NC) with a pre-specified significance level of 95%. Data are presented as the mean ± standard error of the mean (SEM).
Reporting summary
Farther information on inquiry design is available in the Nature Research Reporting Summary linked to this article.
Data availability
The datasets generated during and/or analyzed during the current study are bachelor from the corresponding author on reasonable request. Sequence information that back up the findings of this study are deposited in Gene Expression Omnibus nether the accession code GSE188399.
References
-
Lundborg, Thousand. A 25-year perspective of peripheral nerve surgery: evolving neuroscientific concepts and clinical significance. J. Hand Surg. Am. 25, 391–414 (2000).
-
Evans, P. J., Midha, R. & Mackinnon, South. E. The peripheral nerve allograft: a comprehensive review of regeneration and neuroimmunology. Prog. Neurobiol. 43, 187–233 (1994).
-
Seddon, R. J. Surgical experiences with peripheral nerve injuries. Q. Bull. Northwest Univ. Med. Sch. 21, 201–210 (1947).
-
Daly, W., Yao, L., Zeugolis, D., Windebank, A. & Pandit, A. A biomaterials approach to peripheral nervus regeneration: bridging the peripheral nervus gap and enhancing functional recovery. J. R. Soc. Interface ix, 202–221 (2012).
-
Isaacs, J. Treatment of acute peripheral nerve injuries: current concepts. J. Hand Surg. Am. 35, 491–497 (2010). quiz 498.
-
Strauch, R. J. & Strauch, B. Nerve conduits: an update on tubular nervus repair and reconstruction. J. Mitt Surg. Am. 38, 1252–1255 (2013). quiz 1255.
-
Pindrik, J., Chhabra, A. & Belzberg, A. J. Update on peripheral nerve surgery. Neurosurgery lx, 70–77 (2013).
-
Grayson, West. L. et al. Stromal cells and stem cells in clinical os regeneration. Nat. Rev. Endocrinol. 11, 140 (2015).
-
Bussolati, B. & Camussi, One thousand. Therapeutic utilize of human renal progenitor cells for kidney regeneration. Nat. Rev. Nephrol. eleven, 695 (2015).
-
Assinck, P., Duncan, G. J., Hilton, B. J., Plemel, J. R. & Tetzlaff, W. Prison cell transplantation therapy for spinal cord injury. Nat. Neurosci. xx, 637 (2017).
-
Sakai, D. & Andersson, K. B. J. Stem prison cell therapy for intervertebral disc regeneration: obstacles and solutions. Nat. Rev. Rheumatol. 11, 243 (2015).
-
Behfar, A., Crespo-Diaz, R., Terzic, A. & Gersh, B. J. Cell therapy for cardiac repair—lessons from clinical trials. Nat. Rev. Cardiol. eleven, 232 (2014).
-
Ellis, C., Ramzy, A. & Kieffer, T. J. Regenerative medicine and cell-based approaches to restore pancreatic function. Nat. Rev. Gastroenterol. Hepatol. 14, 612 (2017).
-
Gersey, Z. C. et al. First man feel with autologous Schwann cells to supplement sciatic nervus repair: report of two cases with long-term follow-up. Neurosurg. Focus 42, E2 (2017).
-
Rodrı́Guez, F. J., Verdú, E., Ceballos, D. & Navarro, 10. Nerve guides seeded with autologous schwann cells ameliorate nerve regeneration. Exp. Neurol. 161, 571–584 (2000).
-
Levi, A. D. O. et al. The role of cultured schwann cell grafts in the repair of gaps within the peripheral nervous system of primates. Exp. Neurol. 143, 25–36 (1997).
-
Jesuraj, N. J. et al. Schwann cells seeded in acellular nerve grafts improve functional recovery. Muscle Nerve 49, 267–276 (2014).
-
Hess, J. R. et al. Employ of common cold-preserved allografts seeded with autologous Schwann cells in the treatment of a long-gap peripheral nervus injury. Plast. Reconstr. Surg. 119, 246–259 (2007).
-
Wang, D. et al. Repairing large radial nervus defects by acellular nerve allografts seeded with autologous bone marrow stromal cells in a monkey model. J. Neurotrauma 27, 1935–1943 (2010).
-
Endo, T., Kadoya, K., Suzuki, Y., Kawamura, D. & Iwasaki, Northward. A novel experimental model to make up one's mind the axon-promoting effects of grafted cells subsequently peripheral nervus injury. Front. Jail cell Neurosci. xiii, 280 (2019).
-
McDonald, D., Cheng, C., Chen, Y. & Zochodne, D. Early on events of peripheral nerve regeneration. Neuron Glia Biol. 2, 139–147 (2006).
-
Wakao, Southward., Matsuse, D. & Dezawa, M. Mesenchymal stalk cells as a source of Schwann cells: their anticipated use in peripheral nerve regeneration. Cells Tissues Organs 200, 31–41 (2014).
-
Ma, Thou. S., Boddeke, E. & Copray, S. Pluripotent stalk cells for Schwann cell technology. Stem Cell Rev. xi, 205–218 (2015).
-
Pan, Y. & Cai, S. Current state of the evolution of mesenchymal stem cells into clinically applicative Schwann cell transplants. Mol. Cell Biochem. 368, 127–135 (2012).
-
Walsh, S., Biernaskie, J., Kemp, Southward. W. P. & Midha, R. Supplementation of acellular nerve grafts with skin derived precursor cells promotes peripheral nervus regeneration. Neuroscience 164, 1097–1107 (2009).
-
Bhangra, 1000. South., Busuttil, F., Phillips, J. B. & Rahim, A. A. Using stem cells to grow bogus tissue for peripheral nervus repair. Stalk Cells Int. 2016, ane–18 (2016).
-
Kitada, M., Murakami, T., Wakao, Southward., Li, Chiliad. & Dezawa, M. Directly conversion of adult homo pare fibroblasts into functional Schwann cells that reach robust recovery of the severed peripheral nerve in rats. Glia https://doi.org/10.1002/glia.23582 (2019).
-
Jessen, K. R. & Mirsky, R. Schwann prison cell precursors; multipotent glial cells in embryonic nerves. Front. Mol. Neurosci. https://doi.org/ten.3389/fnmol.2019.00069 (2019).
-
Davies, J. E. et al. Astrocytes derived from glial-restricted precursors promote spinal cord repair. J. Biol. 5, vii (2006).
-
Kadoya, K. et al. Spinal cord reconstitution with homologous neural grafts enables robust corticospinal regeneration. Nat. Med. 22, 479–487 (2016).
-
Jessen, K. R. & Mirsky, R. The repair Schwann prison cell and its part in regenerating nerves. J. Physiol. 594, 3521–3531 (2016).
-
Bosse, F., Hasenpusch-Theil, K., Kury, P. & Muller, H. W. Gene expression profiling reveals that peripheral nerve regeneration is a event of both novel injury-dependent and reactivated developmental processes. J. Neurochem. 96, 1441–1457 (2006).
-
Jessen, Thousand. R. & Mirsky, R. Negative regulation of myelination: relevance for development, injury, and demyelinating disease. Glia 56, 1552–1565 (2008).
-
Jessen, K. R. & Mirsky, R. The origin and development of glial cells in peripheral nerves. Nat. Rev. Neurosci. 6, 671–682 (2005).
-
Jessen, K. R., Mirsky, R. & Lloyd, A. C. Schwann cells: development and role in nervus repair. Cold Bound Harb. Perspect. Biol. 7, a020487 (2015).
-
Kuhlbrodt, K., Herbarth, B., Sock, E., Hermans-Borgmeyer, I. & Wegner, M. Sox10, a novel transcriptional modulator in glial cells. J. Neurosci. 18, 237–250 (1998).
-
Liu, Z. et al. Specific marking expression and prison cell state of Schwann cells during culture in vitro. PLoS I 10, e0123278 (2015).
-
Jessen, One thousand. R. et al. The Schwann cell precursor and its fate: a written report of jail cell expiry and differentiation during gliogenesis in rat embryonic fretfulness. Neuron 12, 509–527 (1994).
-
Bornstein, Due south. R., Berger, I. & Steenblock, C. Are Nestin-positive cells responsive to stress? Stress 23, 662–666 (2020).
-
Stratton, J. A. et al. Purification and characterization of schwann cells from adult human pare and nervus. eNeuro https://doi.org/10.1523/ENEURO.0307-16.2017 (2017).
-
Kumar, R. et al. Adult skin-derived forerunner Schwann cells exhibit superior myelination and regeneration supportive properties compared to chronically denervated nerve-derived Schwann cells. Exp. Neurol. 278, 127–142 (2016).
-
Jessen, Thousand. R. & Mirsky, R. Origin and early evolution of Schwann cells. Microsc. Res. Tech. 41, 393–402 (1998).
-
Endo, T., Kadoya, K., Kawamura, D. & Iwasaki, N. Evidence for jail cell-contact factor interest in neurite outgrowth of dorsal root ganglion neurons stimulated by Schwann cells. Exp. Physiol. 104, 1447–1454 (2019).
-
Jessen, K. R. & Arthur-Farraj, P. Repair Schwann cell update: adaptive reprogramming, EMT, and stemness in regenerating fretfulness. Glia 67, 421–437 (2019).
-
Wanner, I. B. et al. Invariant mantling of growth cones by Schwann cell precursors characterize growing peripheral nervus fronts. Glia 54, 424–438 (2006).
-
Stratton, J. A. et al. Macrophages regulate schwann cell maturation subsequently nerve injury. Cell Rep. 24, 2561–2572.e2566 (2018).
-
Dun, Ten. P. et al. Macrophage-derived Slit3 controls prison cell migration and axon pathfinding in the peripheral nerve span. Prison cell Rep. 26, 1458–1472.e1454 (2019).
-
Arthur-Farraj, P. J. et al. Changes in the coding and non-coding transcriptome and Dna methylome that ascertain the Schwann prison cell repair phenotype later on nerve injury. Cell Rep. 20, 2719–2734 (2017).
-
Aquino, J. B. Schwann cell precursors in health and disease. Glia 66, 465–476 (2018).
-
Grigoryan, T. & Birchmeier, W. Molecular signaling mechanisms of axon-glia communication in the peripheral nervous system. Bioessays 37, 502–513 (2015).
-
Arthur-Farraj, P. J. et al. c-Jun reprograms Schwann cells of injured fretfulness to generate a repair cell essential for regeneration. Neuron 75, 633–647 (2012).
-
Fontana, X. et al. c-Jun in Schwann cells promotes axonal regeneration and motoneuron survival via paracrine signaling. J. Cell Biol. 198, 127–141 (2012).
-
Fu, S. Y. & Gordon, T. The cellular and molecular basis of peripheral nerve regeneration. Mol. Neurobiol. 14, 67–116 (1997).
-
Lindsay, R. M. Nerve growth factors (NGF, BDNF) enhance axonal regeneration simply are not required for survival of adult sensory neurons. J. Neurosci. 8, 2394–2405 (1988).
-
Kotton, D. Northward. & Morrisey, Eastward. E. Lung regeneration: mechanisms, applications and emerging stem jail cell populations. Nat. Med. 20, 822 (2014).
-
Chichagova, V. et al. Cellular regeneration strategies for macular degeneration: past, present and hereafter. Centre https://doi.org/10.1038/s41433-018-0061-z (2018).
-
Zhong, J., Wang, Due south., Shen, Due west.-B., Kaushal, S. & Yang, P. The current condition and futurity of cardiac stem/progenitor cell therapy for congenital middle defects from diabetic pregnancy. Pediatr. Res. 83, 275–282 (2018).
-
Fischer, I., Dulin, J. N. & Lane, Grand. A. Transplanting neural progenitor cells to restore connectivity after spinal string injury. Nat. Rev. Neurosci. 21, 366–383 (2020).
-
Rickmann, Chiliad., Fawcett, J. & Keynes, R. The migration of neural crest cells and the growth of motor axons through the rostral half of the chick somite. Development 90, 437–455 (1985).
-
Grim, Chiliad., Halata, Z. & Franz, T. Schwann cells are not required for guidance of motor nerves in the hindlimb in Splotch mutant mouse embryos. Anat. Embryol. 186, 311–318 (1992).
-
Jessen, K. R., Mirsky, R. & Arthur-Farraj, P. The office of cell plasticity in tissue repair: adaptive cellular reprogramming. Dev. Prison cell 34, 613–620 (2015).
-
Blanpain, C. & Fuchs, East. Plasticity of epithelial stem cells in tissue regeneration. Science 344, 1242281–1242281 (2014).
-
Raff, One thousand. Adult stem jail cell plasticity: fact or antiquity? Annu. Rev. Prison cell Dev. Biol. 19, 1–22 (2003).
-
Li, L. & Xie, T. STEM CELL NICHE: structure and function. Annu. Rev. Cell Dev. Biol. 21, 605–631 (2005).
-
Lane, S. W., Williams, D. A. & Watt, F. M. Modulating the stem prison cell niche for tissue regeneration. Nat. Biotechnol. 32, 795–803 (2014).
-
Meyer, D. & Birchmeier, C. Multiple essential functions of neuregulin in development. Nature 378, 386–390 (1995).
-
Grinspan, J. B., Marchionni, Grand. A., Reeves, One thousand., Coulaloglou, M. & Scherer, S. S. Axonal interactions regulate schwann cell apoptosis in developing peripheral nervus: neuregulin receptors and the role of neuregulins. J. Neurosci. sixteen, 6107 (1996).
-
Fricker, F. R. et al. Axonally derived neuregulin-1 is required for remyelination and regeneration after nerve injury in adulthood. J. Neurosci. 31, 3225–3233 (2011).
-
Stassart, R. M. et al. A function for Schwann cell–derived neuregulin-1 in remyelination. Nat. Neurosci. https://www.nature.com/manufactures/nn.3281#supplementary-information (2012).
-
Allodi, I., Udina, E. & Navarro, X. Specificity of peripheral nervus regeneration: Interactions at the axon level. Prog. Neurobiol. 98, 16–37 (2012).
-
Kadoya, K. et al. Combined intrinsic and extrinsic neuronal mechanisms facilitate bridging axonal regeneration one year later spinal string injury. Neuron 64, 165–172 (2009).
-
Painter, Thousand. W. et al. Diminished Schwann cell repair responses underlie historic period-associated impaired axonal regeneration. Neuron 83, 331–343 (2014).
-
Brosius Lutz, A. & Barres, B. A. Contrasting the glial response to axon injury in the fundamental and peripheral nervous systems. Dev. Jail cell 28, vii–17 (2014).
-
Mar, F. Thousand., Bonni, A. & Sousa, G. M. Cell intrinsic control of axon regeneration. EMBO Rep. xv, 254 (2014).
-
Riethmacher, D. et al. Astringent neuropathies in mice with targeted mutations in the ErbB3 receptor. Nature 389, 725 (1997).
-
Nieuwenhuis, B., Haenzi, B., Andrews, M. R., Verhaagen, J. & Fawcett, J. W. Integrins promote axonal regeneration later on injury of the nervous arrangement. Biol. Rev. Camb. Philos. Soc. 93, 1339–1362 (2018).
-
Ide, C., Tohyama, M., Yokota, R., Nitatori, T. & Onodera, S. Schwann jail cell basal lamina and nerve regeneration. Brain Res. 288, 61–75 (1983).
-
Nieuwenhuis, B., Haenzi, B., Andrews, Chiliad. R., Verhaagen, J. & Fawcett, J. W. Integrins promote axonal regeneration later on injury of the nervous system. Biol. Rev. 93, 1339–1362 (2018).
-
Chen, Z. L. & Strickland, S. Laminin gamma1 is disquisitional for Schwann cell differentiation, axon myelination, and regeneration in the peripheral nerve. J. Jail cell Biol. 163, 889–899 (2003).
-
Isaacman-Beck, J., Schneider, 5., Franzini-Armstrong, C. & Granato, M. The lh3 glycosyltransferase directs target-selective peripheral nervus regeneration. Neuron 88, 691–703 (2015).
-
Sock, E. & Wegner, K. Transcriptional control of myelination and remyelination. Glia 67, 2153–2165 (2019).
-
Woodhoo, A. et al. Notch controls embryonic Schwann cell differentiation, postnatal myelination and adult plasticity. Nat. Neurosci. 12, 839–847 (2009).
-
Stoll, K., Griffin, J. W., Li, C. Y. & Trapp, B. D. Wallerian degeneration in the peripheral nervous system: participation of both Schwann cells and macrophages in myelin degradation. J. Neurocytol. eighteen, 671–683 (1989).
-
Boissonnas, A. et al. Imaging resident and recruited macrophage contribution to Wallerian degeneration. J. Exp. Med. https://doi.org/10.1084/jem.20200471 (2020).
-
Lindborg, J. A., Mack, K. & Zigmond, R. E. Neutrophils are disquisitional for myelin removal in a peripheral nerve injury model of Wallerian degeneration. J. Neurosci. https://doi.org/10.1523/jneurosci.2085-17.2017 (2017).
-
Kalinski, A. L. et al. Analysis of the Immune Response to Sciatic Nerve Injury Identifies Efferocytosis equally a Key Mechanism of Nervus Debridement (Common cold Spring Harbor Laboratory, 2022).
-
Gomez-Sanchez, J. A. et al. Afterward nerve injury, lineage tracing shows that myelin and remak Schwann cells elongate extensively and branch to form repair schwann cells, which shorten radically on remyelination. J. Neurosci. 37, 9086–9099 (2017).
-
Mirsky, R. & Jessen, K. R. Isolation of Schwann cell precursors from rodents. Methods Mol. Biol. 1739, iii–fifteen (2018).
-
Kaewkhaw, R., Scutt, A. M. & Haycock, J. West. Integrated culture and purification of rat Schwann cells from freshly isolated developed tissue. Nat. Protoc. vii, 1996–2004 (2012).
-
Maurel, P. Preparation of neonatal rat schwann cells and embryonic dorsal root ganglia neurons for in vitro myelination studies. Methods Mol. Biol. https://doi.org/10.1007/978-i-4939-7649-2_2 (2018).
-
Schneider, C. A., Rasband, W. S. & Eliceiri, G. W. NIH Image to ImageJ: 25 years of image analysis. Nature Methods 9, 671, https://doi.org/10.1038/nmeth.2089 (2012).
-
Meijering, Due east. et al. Design and validation of a tool for neurite tracing and assay in fluorescence microscopy images. Cytometry Function A 58, 167–176 (2004).
-
Omura, T. et al. Robust Axonal Regeneration Occurs in the Injured Bandage/Ei Mouse CNS. Neuron 86, 1215–1227, https://doi.org/10.1016/j.neuron.2015.05.005 (2015).
-
Trapnell, C. et al. Differential gene and transcript expression assay of RNA-seq experiments with TopHat and Cufflinks. Nat. Protoc. 7, 562 (2012).
Acknowledgements
This study was supported by the Nippon Orthopaedic and Traumatology Research Foundation, the Full general Insurance Association of Japan, a Grant-in-Aid for Scientific Inquiry (JP17K10914), and the Japan Agency for Medical Enquiry and Development (JP21gm6210004), the Kobayashi Foundation, and the SEI Grouping CSR Foundation. We are thankful to Thousand. Endo for her assistance, National BioResource Project-Rat (http://www.anim.med.kyoto-u.air-conditioning.jp/NBR/) for providing LEW-Tg(Gt(ROSA)26Sor-DsRed*)7Jmck rat (#0404), and the Open Facility, Hokkaido University Sousei Hall for giving access to their confocal microscopy and cryostat.
Writer information
Affiliations
Contributions
T.E. and K.K. designed the enquiry. T.East. conducted the research. T.E., Yard.Thousand., T.South., and 1000.A.T. analyzed the data. N.I. supervised the project. Y.Southward. and D.K. contributed with unpublished reagents and analytic tools. T.Eastward. and M.K. wrote the paper.
Corresponding writer
Ideals declarations
Competing interests
The authors declare no competing interests.
Additional information
Publisher'southward note Springer Nature remains neutral with regard to jurisdictional claims in published maps and institutional affiliations.
Supplementary information
Rights and permissions
Open up Admission This article is licensed under a Creative Commons Attribution 4.0 International License, which permits use, sharing, accommodation, distribution and reproduction in any medium or format, as long as y'all give advisable credit to the original author(s) and the source, provide a link to the Creative Commons license, and indicate if changes were made. The images or other third party material in this article are included in the commodity's Creative Commons license, unless indicated otherwise in a credit line to the textile. If material is non included in the article's Creative Commons license and your intended use is not permitted by statutory regulation or exceeds the permitted employ, you will need to obtain permission direct from the copyright holder. To view a copy of this license, visit http://creativecommons.org/licenses/by/iv.0/.
Reprints and Permissions
About this article
Cite this article
Endo, T., Kadoya, K., Suzuki, T. et al. Mature merely not developing Schwann cells promote axon regeneration after peripheral nervus injury. npj Regen Med 7, 12 (2022). https://doi.org/ten.1038/s41536-022-00205-y
-
Received:
-
Accepted:
-
Published:
-
DOI : https://doi.org/x.1038/s41536-022-00205-y
How Do Schwann Cells Repair Damaged Nerves,
Source: https://www.nature.com/articles/s41536-022-00205-y
Posted by: wilsonfroce1940.blogspot.com
0 Response to "How Do Schwann Cells Repair Damaged Nerves"
Post a Comment